Raman Spectroscopy
If a beam of light is passed through a transparent substance, a small part of the radiation energy is scattered, this scattering persisting even if all dust particles are rigorously excluded from the substance. If the very narrow frequency band radiation or monochromatic radiation is used, the scattered energy will consist almost entirely of radiation of the incident frequency (Rayleigh scattering) but, in addition, certain distance frequencies above and below that of the incident beam will be scattered, this is referred to as Raman scattering.
Quantum Theory of Raman Scattering
Raman spectroscopy deals with the scattering of light and not with its absorption, like other conventional branches of spectroscopy. Consider a photon of frequency v falling on a molecule, if there is an elastic collision, then the scattered photon will have the same energy as the incident photon if there is an inelastic collision, the scattered photon will have either a higher or a lower energy than the incident photon. If we assume that the kinetic energy of both that is the photon and the molecule remains unchanged before and after the collision, then we write from the law of conservation of energy:
hv + E = hv' + E' ----- (1)
Where hv is the energy of incident photon before the collision and hv' is the energy of the scattered photon after the collision, E is the molecular energy before the collision, and E' is the energy of the molecule after the collision. Rearranging equation 1, we get ----
(E'-E)/h = v-v' ----- (2)
Now consider the following cases :
Case I. v=v' so that E=E'
Case II(a). v<v' so that E>E'
Case II(b). v>v' so that E<E'
Here case I is referred to as Rayleigh scattering, while the cases II(a) and II(b) referred to Raman scattering. In the case of Rayleigh scattering, the frequency of the scattered photon and the frequency of the incident photon is the same, while in the case of Raman scattering, when the collision occurred between the photon and the molecule there are two possibilities, one is energy from photon transferred to molecule and other is energy taken away from the molecule. The Rayleigh scattering and the Raman scattering are schematically represented as:
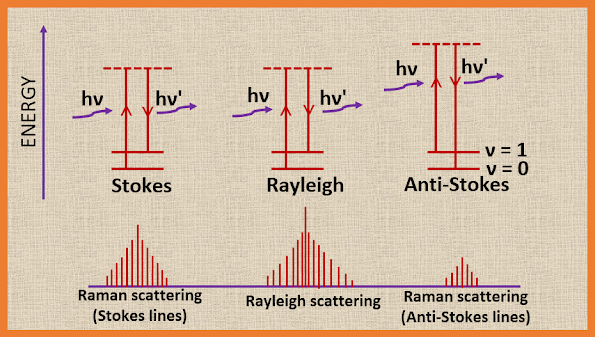 |
The Rayleigh scattering and the Raman scattering |
If the molecule, excited to the higher unstable state when the molecule returns to the original vibrational state, we get Rayleigh scattering. If the molecule returns to a different vibrational state, we get Raman scattering (stokes lines). If the molecule, initially in the first excited vibrational state, excited to the higher unstable vibrational state, and finally returns to the ground state, this again gives rise to Raman scattering (anti-Stokes lines). Thus, the Raman spectrum of a molecule consists of Stokes lines and anti-Stokes lines, situated symmetrically about the Rayleigh line.
The Rayleigh line is far more intense than the Stokes line, and the Stokes line has a greater intensity than the anti-Stokes lines. The anti-Stokes lines are very difficult to observe in conventional Raman spectroscopy because these molecules are returned from an unstable excited vibrational state to the ground state and there are initially very few molecules in the excited vibrational state.
Classical Theory of Raman Scattering
When a molecule is put into a static electric field it suffers some distortion, the negative pole attracted the positively charged nuclei, and the positive pole attracted the electrons. This separation of charge centres causes an induced electric dipole moment to be set up in the molecule and the molecule is said to be polarized. The size of the induced dipole µ, depends both on the magnitude of the applied field, E, and on the case with which the molecule can be distorted. We can write
µ = αE ----- (3)
Where α is the polarizability of the molecule. The electric field vector E itself is given by:
E = E0 sin 2πνt ----- (4)
Where E0 is the amplitude of the vibrating electric field vector and v is the frequency of of the incident light radiation. Thus from equation (3) and (4), we get
µ = αE0 sin 2πνt ----- (5)
Such an oscillating dipole emits radiation of its own oscillation with a frequency v, giving the Rayleigh scattered beam, if , however, the polarizability varies slightly with molecular vibration, we can write
α = α0 +
(Әα/Әq) q ----- (6)
Where the coordinate q describe the molecular vibration. We can also write q as
q =
q0 sin 2πνmt ----- (7)
Where q0
is the amplitude of the molecular vibration and vm
is its frequency. From equation (6) and (7), we have
α = α0 +
(Әα/Әq)
q0 sin 2πνmt ----- (8)
Substituting for α in equation (5), we have
µ = α0E0 sin 2πνt + (Әα/Әq) q0E0
sin 2πνt sin 2πνmt ----- (9)
Making use of trigonometric relation :
sin x sin y = 1/2 [cos (x-y) - cos (x+y)]
This equation reduces to
µ = α0E0
sin 2πνt + 1/2 (Әα/Әq) q0E0 cos 2π (ν-νm) t – 1/2
(Әα/Әq) q0E0 cos 2π
(ν +νm) t ----- (10)
= α0E0 sin 2πνt + 1/2 (Әα/Әq) q0E0
x [cos 2π (ν-νm) t – cos 2π (ν+νm) t] ----- (11)
Thus the oscillating dipole has three distinct frequency components : (i) the exciting frequency v with amplitude α0E0 (ii) v-vm
and (iii) v+vm with very small amplitudes = (1/2) (Әα/Әq) q0E0. Hence, the Raman spectrum of a vibrating molecule consists of a relatively intense band at the incident frequency and two very weak bands at frequencies slightly above and below that of the intense band.
If, however, the molecular vibration does not change the polarizability of the molecule, then (Әα/Әq) = 0 so that the dipole oscillates only at the frequency of the incident (exciting) radiation. The same is true of the molecular rotation. We conclude that for a molecular vibration or rotation to be active in the Raman spectrum, it must cause a change in the molecular polarizability, i.e., Әα/Әq ≠ 0. --- (12)
Homonuclear diatomic molecules such as H2, N2, O2, which do not show IR spectra because they do not possess a permanent dipole moment, do show Raman spectra since their vibration is accompanied by a change in polarizability of the molecule. As a consequence of the change in polarizability, there occurs a change in the induced dipole moment at the vibrational frequency.
Rotational Raman Spectra
For the vibrational Raman spectrum of a diatomic molecule, the selection rule is
∆ν = ±1 ----- (13)
For the rotational Raman spectrum of a diatomic molecule, the selection rule is
∆J = 0, ±2 ----- (14)
For the rotational selection rule, the operative part is
∆J = +2 ----- (15)
Since ∆J = 0 corresponds to Rayleigh scattering and ∆J = -2 transition can be ignored as the rotational quantum number of the upper state must be greater than that of the lower state. Using the selection rule with the energy level expression for the rigid diatomic rotor, viz,
F(J) = BJ (J+1) cm-1 (J = 0, 1, 2, 3 ---)
We find that ∆F(J) = F(J+2) - F(J) = B (4J+1) cm-1
Since ∆J = +2, we may level these lines S branch lines and thus,
∆F(J) = B(4J+6) cm-1 (J = 0, 1, 2, 3 ---) --- (16)
Where J is the rotational quantum number in the lower state.
Thus if the rotational energy gains by the molecule from the photon during collision we have a series of S branch lines to the low wavenumber side of the exciting line (Stokes line), while if the molecule loses energy to the photon the S branch lines appear on the high wavenumber side (anti-Stokes lines). The wavenumber of the corresponding spectral lines are given by :
ν̅s = ν̅exc ± ∆F (J) = ν̅exc ± B
(4J +6) cm-1 ----- (17)
Where the plus(+) sign refers to anti-Stokes lines, the minus (-) sign to Stokes lines, and ν̅exc is the wavenumber of the exciting radiation.
The energy level diagram and the rotational Raman spectrum for a diatomic molecule are shown in the figure. We see that the first Stokes line (or the first anti-stokes line) appears at a distance of 6B from the exciting Rayleigh line. This is evident from equation 16, where putting J=0 we obtain ∆F (J) = 6B. The separation of the successive Raman lines is, however, 4B, as can be easily verified by putting J = 1, 2, 3, etc. in equation 17.
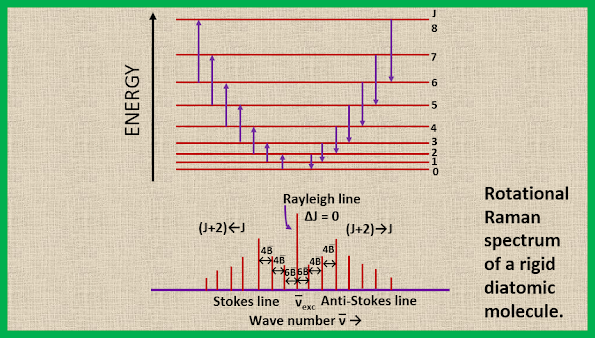 |
Rotational Raman spectrum of a rigid diatomic molecule |
Rotation-Vibration Raman Spectrum
For the rotation-vibration Raman spectrum, the selection rules involve the changes in both the vibrational and the rotational quantum numbers. For the rotation vibration Raman spectrum of a diatomic molecule the selection rules are:
∆ν = +1;
∆J = 0, ±2 ----- (18)
Since at room temperature most of the molecules are in the ground vibrational state (v = 0), so we see, only the vibrational transition from v = 0 to v = 1. The transition with ∆J = 0 form a Q-branch, the transition with ∆J = +2 form an S-branch, and the transition with ∆J = -2 form an O-branch. The rotational Raman transitions accompanying a 0→1 vibrational transition are shown in the figure. Here, ∆ν̅ measures the displacement from the exciting mercury line. The Q-branch exhibits an intense narrow line at ν̅exc ± B that is usually unresolved while the S and O branches form weak wings which extend to lower and higher wave numbers, respectively, from the intense narrow line.
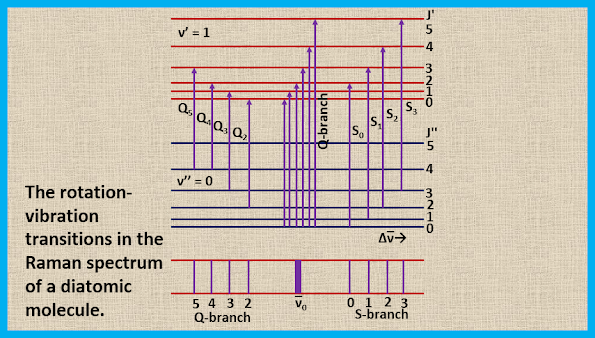 |
The rotation-vibration transitions in the Raman spectrum of a diatomic molecule |